by Gertrud U. Rey
It is now a little more than a year since the emergence of SARS-CoV-2, and we already have several highly effective vaccines against this virus. Because of my previous research experience in vaccine science, I was very skeptical about the promise of a SARS-CoV-2 vaccine this soon. I was wrong, and I could not be happier about that.
Two of the leading vaccines were developed by Pfizer/BioNTech and Moderna and consist of a messenger RNA (mRNA) that encodes the full-length SARS-CoV-2 spike protein. Upon injection into a vaccine recipient, the mRNA would enter cells and be translated by the host protein synthesis machinery into the SARS-CoV-2 spike protein, which would then serve as an antigen to promote an immune response. mRNA vaccines are non-infectious and do not integrate into the genome, meaning that there is no risk of infection or mutations caused by inserted vaccine sequences. Although these vaccines are the first of their kind to be licensed for widespread use, the concept is not new. Reports of the first successful translation of a foreign mRNA in animals were published in 1990, and this technology has been refined ever since. Progress in the field has been hampered by concerns that the inherent instability of RNA would prevent its use for delivery as a therapeutic or vaccine. However, research has shown that the stability of RNA can be increased through various modifications and delivery methods.
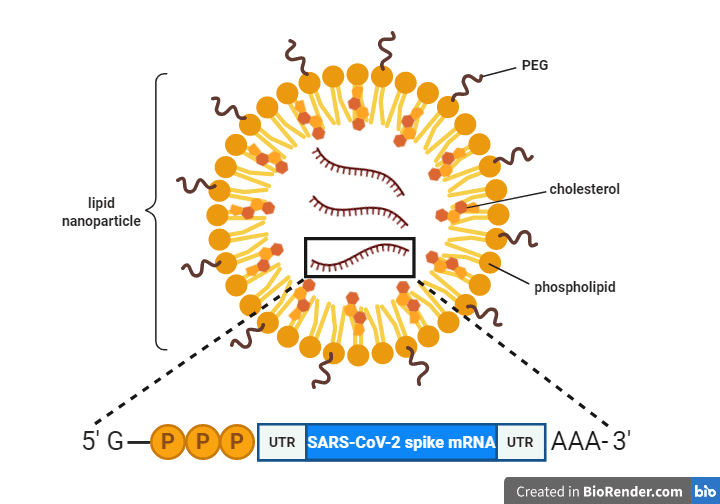
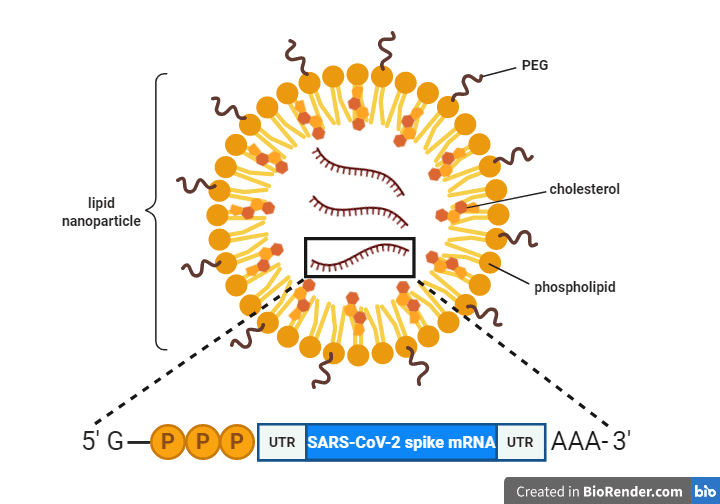
One way a vaccine mRNA molecule can be modified is by placing it between two RNA sequences that don’t code for protein, i.e., untranslated regions (UTRs; see graphic), which stabilize the mRNA and optimize it for translation. The ends of the mRNA – also known as the 5′ and 3′ ends, respectively – can be further modified by addition of a “cap” and a “poly(A) tail.” The cap consists of a modified guanosine nucleotide followed by three phosphates (“G-PPP” in the graphic) and serves as a recognition signal for the cellular ribosome to bind and translate the mRNA. The poly(A) tail is a string of adenosine nucleotides (“AAA” in the graphic), which further stabilize the mRNA.
A common method for encapsulating and delivering the mRNA into cells is to encase it in a cocoon of phospholipids. For example, the mRNA molecule in both the Pfizer and Moderna vaccines is encapsulated in a lipid nanoparticle (pictured), which protects the mRNA from degradation and ensures proper delivery into cells. Addition of cholesterol molecules makes the nanoparticle more fluid and is thought to increase its ability to fuse with our cell’s membranes to deliver the mRNA into our cells. Addition of polyethylene glycol (PEG) increases the potency of the vaccine particle by hiding it from the host immune system, making it more water soluble, and slowing its degradation.
One of the reasons why SARS-CoV-2 mRNA vaccines could be produced so quickly is because all this basic science was already in place at the start of the pandemic. And although the SARS-CoV-2 vaccines are the first mRNA vaccines to be authorized by the FDA for emergency use, several mRNA vaccines have undergone clinical trials in humans before, for at least four infectious diseases: rabies, influenza, cytomegalovirus infection, and Zika virus infection.
Another factor that helped speed up the process of SARS-CoV-2 vaccine production is that, luckily, scientists were able to extrapolate the insight gained from the study of other coronaviruses to SARS-CoV-2. Like the spike protein of other coronaviruses, the SARS-CoV-2 spike protein is highly immunogenic and is targeted by neutralizing antibodies, which bind viral antigens to inactivate the virus and prevent infection of new cells. The spike protein also mediates binding of the virus to the ACE2 host cell receptor via spike’s receptor-binding domain and fusion of the viral particle with the host cell membrane via spike’s fusion domain. However, to mediate this fusion, the SARS-CoV-2 spike protein undergoes a structural rearrangement from its pre-fusion conformation. By 2017, scientists at the Vaccine Research Center of the National Institute of Allergy and Infectious Diseases had already determined that the pre-fusion form of Middle East respiratory syndrome coronavirus (MERS-CoV) is more immunogenic than its post-fusion form. Accordingly, they had spent several years engineering a mutation that locks the translated spike protein into its pre-fusion structure. When the SARS-CoV-2 genome sequence was published one year ago, scientists were able to compare it to the MERS-CoV sequence and identify the exact location where the pre-fusion stabilizing mutation had to be made. And luckily, making the mutation in the SARS-CoV-2 spike mRNA sequence stabilized the spike protein in its pre-fusion conformation.
Conventional vaccine strategies have repeatedly failed to yield vaccines against challenging viruses like HIV-1, herpes simplex virus, and respiratory syncytial virus (RSV), while recent advances in mRNA vaccine technology show promise in immunizing against some of these viruses. For example, RSV poses a substantial public health threat due to its association with severe morbidity and mortality in infants and premature babies. Despite 60 years of continual efforts, we still don’t have a licensed RSV vaccine, in part because natural RSV infection does not induce a durable immune response. We do know that the RSV F (fusion) protein is highly conserved and elicits broadly neutralizing antibodies, and recent studies have shown that similar to the case of the SARS-CoV-2 spike protein, most neutralizing activity in human serum is directed against the pre-fusion form of the RSV F protein. This observation inspired scientists at Moderna to develop an RSV pre-fusion F protein mRNA vaccine, with Phase I clinical trial data showing promising results.
One of the exciting features of the mRNA vaccine platform is that it is not only applicable to preventing viral diseases but can also be used for treating cancer. Cancer mRNA vaccines would target tumor-associated antigens that are preferentially expressed in cancer cells.
Vaccination remains one of the most effective public health measures for preventing and controlling infectious diseases. However, conventional vaccine approaches using live-attenuated and inactivated virus vaccines are time-consuming and expensive. mRNA vaccines can be produced more quickly and cost-effectively than conventional vaccines because they obviate the need for growing and/or repeatedly passaging viruses in cell culture. Nonetheless, we would not know any of this without decades of prior studies, which further highlights the importance of regularly funding basic research.
Thank you for this excellent article with a brief history of mRNA vaccine development, and particularly the SARS-CoV-2 vaccine, and how previous scientific breakthroughs assisted with the speedy development of an effective vaccine. The plug for continued basic scientific research cannot be overstated! The author writes eloquently about a subject that many need to be better educated about. It is refreshing to read about the science, written so clearly and simply. The diagram is awesome! Hopefully this piece will be more widely disseminated.
It should be pointed out that an anti-cancer vaccine (against prostate cancer) trial has already been conducted by Curevac. While the initial trials did give encouraging results, I believe it unfortunately failed in phase III.
“Addition of polyethylene glycol (PEG) increases the potency of the vaccine particle by hiding it from the host immune system, making it more water soluble, and slowing its degradation.”
Hmm: Good thing the viruses didn’t come up with lipid nanoparticles first. They could have got into cells without needing a key, or any other fancy proteins. Now one has to ask: can viruses code for coating in their own lipid ‘cloaks of invisibility’?
Hope not. Eh!
Dear Dctor.
Could you tell me what are the possible consequences of using an mRNA vaccine on an individual with active retrovirus infection?
Dr Martins. O.P.
What limits the production of spike proteins?
Will a single RNA strand encoding a spike be translated repeatedly? If so, what puts limits on the translation process?
Thank you for your insights
An overview article (pre-corona) lists quitte some of the achievements upto 2018 on mRNA vaccines:
https://www.nature.com/articles/nrd.2017.243
Furthermore, the vaccines by J&J and Oxford-AstraZeneca, are not that different. They use a carrier virus to bring DNA into the cell, which gets transcribed to RNA and then to Spike. The advantage is the fact that DNA is more stable, hence the easier storage.
Many PhD’s can be performed on the difference in vaccines and the cause of these. An interesting time with many different candidates to compare from classical (Sinovac) to mRNA and all in-between (subunit, vector, etc)